Here on Earth, as well as elsewhere throughout the Universe, a tremendous variety of naturally occurring elements can be found. More than 90 species of atom — from hydrogen up through plutonium — have been detected in various environments, but astrophysically, very few events are capable of creating the heaviest elements of all. Where did these heavy elements, including silver, iodine, tungsten, platinum, gold, mercury, uranium, and more, ultimately come from? For all of the 20th century and much of the 2000s, we had only theoretical ideas, not the observational confirmation we desired.
That situation appeared to change in dramatic fashion on August 17, 2017, when we detected the first signals from a pair of merging neutron stars: a cataclysmic, black hole-creating event known as a kilonova. This one neutron star-neutron star merger, occurring in a galaxy just 130 million light-years away and just the 8th gravitational wave event ever observed, seemed to provide the answer. A wide variety of heavy elements were created in this merger and detected in its afterglow, including so much gold that, if we were to place it on a scale, would weigh about twenty times as much as Earth’s Moon.
But now, seven years later, it no longer looks like the amount of gold present in the Universe can be explained by neutron star mergers alone. Here’s why the best idea for the origin of gold simply doesn’t add up.
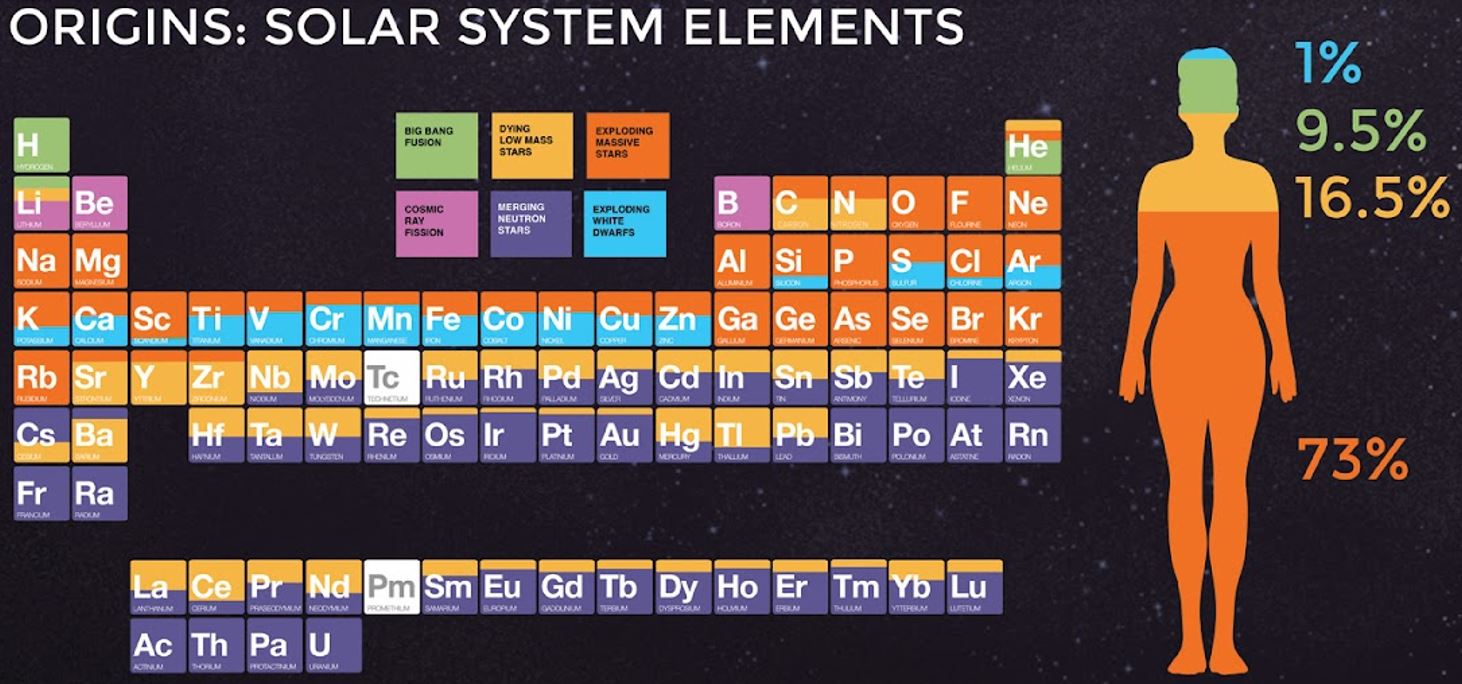
Whenever you build an instrument to probe and investigate the Universe, you inherently make an assumption: that what you observe, over whatever fraction of the Universe (in both time and space) you’re capable of measuring, is a good proxy for the full suite of what is out there in the vastness of the cosmos.
When we look out at the Universe with our telescopes, we don’t need to survey the entire sky; we survey a portion of the sky, and extrapolate what we see in that region to draw inferences about the overall population of objects elsewhere, including where we haven’t looked. Similarly, when we look at a reasonably large region of space for a sufficient duration of time — for an example, something like 8 hours and 46 minutes — we can anticipate what we ought to expect to occur over much longer timescales, like years, decades, centuries, or millennia, by simply extrapolating the observed event rate over the time we did collect our data.
But there’s a flaw to this method. Sometimes, you get lucky: you see a rare event or object because you happened to be looking in the right place at the right time, and you wind up vastly overestimating how frequently such events occur. Sometimes, you get unlucky: you miss relatively common events or objects, because you happened to be looking at a certain place and time where no such events or objects were found. Only with better, more comprehensive data can we account for these potential biases.
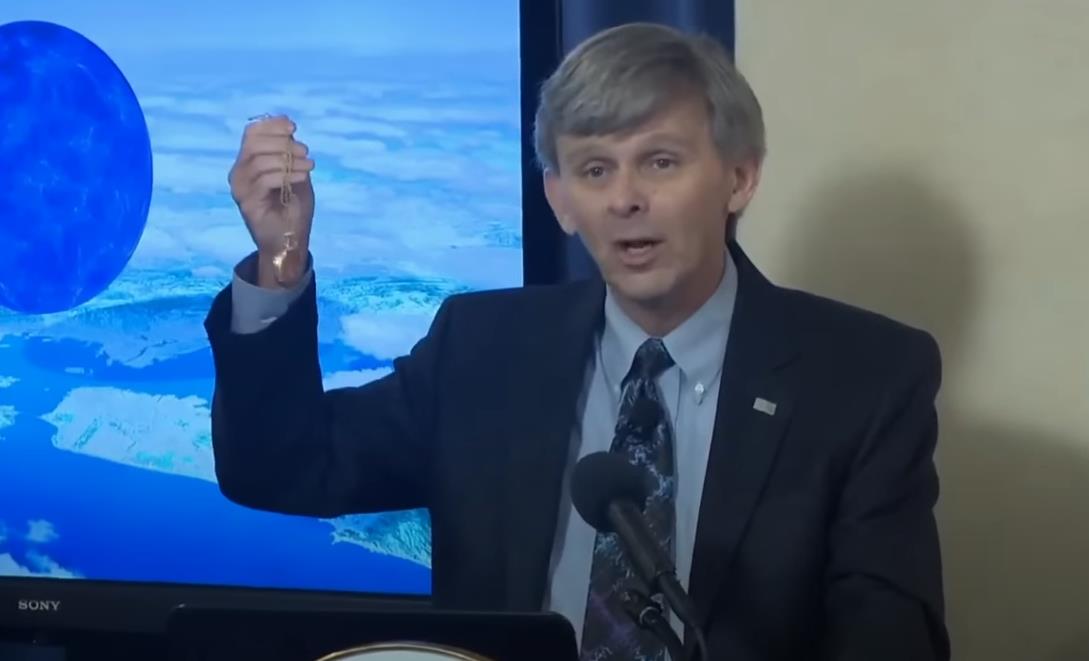
When the event of August 17, 2017 was announced to the world, it was accompanied by a now-famous video where the Executive Director of LIGO, Dr. David Reitze, pulled out his great-grandfather’s gold watch — an artifact that’s now more than a century old itself — and declared that “the gold in this watch was very likely produced in the collision of two neutron stars approximately billions of years ago.” Many other similarly heavy elements, including platinum and uranium, were also produced, creating a total of about 16,000 Earth masses worth of heavy elements: about 5% of the mass of the Sun, from this one event alone.
Based on:
- the length of time that gravitational wave detectors like LIGO and Virgo had been operating,
- the amount of sky coverage those detectors were capable of seeing signals from,
- the sensitivity of those detectors, including how far away from Earth such events could be observed,
- the observed event rates for black hole-black hole mergers, neutron star-neutron star merger, and black hole-neutron star mergers at that moment,
- and the total amounts of heavy elements produced in this kilonova event,
it seemed like the mystery was solved. The Universe’s gold, as well as practically all other heavy elements above zirconium (which is just element #40 on the periodic table), were primarily produced in neutron star-neutron star mergers.

But then a funny thing happened over the next seven years, bringing us up to the present day: only one other neutron star-neutron star merger has ever been detected, and unlike the first event, it did not produce a kilonova or create any heavy elements. Despite several years of additional observing time, significant upgrades to the existing detectors that increase their sensitivity range, and tremendous numbers of black hole-black hole mergers and many black hole-neutron star mergers subsequently observed — bringing the total number of gravitational wave events up to over 100 — that one event from August 17, 2017 remains the only directly observed neutron star-neutron star merger to produce heavy elements.
This has taught us something remarkable and important: our initial estimate, that around 100% of the heaviest elements in the Universe was produced from neutron star-neutron star mergers, is no longer consistent with the data we’ve collected. Something else, beyond what we’ve seen so far, must be at play. Three possible explanations immediately spring to mind, but all three have complicated problems.
- Perhaps the neutron star-neutron star merger rate, creating kilonovae, was greater in the past than it is today.
- Perhaps the brightest, most energetic supernovae create a larger abundance of heavier elements than expected, and these play an important role even for elements heavier than zirconium.
- Or perhaps, during the giant phase of an evolved star’s life, a slow addition of neutrons allows elements to build their way up the periodic table, creating those observed heavy elements.
Each scenario, however, compelling, has its flaws.

Scenario #1: the neutron star-neutron star merger rate evolves, and was larger in the past.
When we look out across the Universe to great cosmic distances, we also look back in time. But when it comes to the detection of neutron star-neutron star mergers, even our most powerful gravitational wave observatories can only detect such events if they’re withing a few hundred million light-years of Earth. Although this encompasses millions of galaxies, and takes us back hundreds of millions of years in cosmic history, we have to remember it’s now been 13.8 billion years since the Big Bang occurred. When it comes to neutron star-neutron star mergers, we’re only sensitive to the ones that have occurred over the past 1-3% of our Universe’s history.
The neutron star-neutron star mergers that do produce kilonovae — i.e., the ones that don’t collapse directly to a black hole without emitting any ejecta — also create short-period gamma-ray bursts, so one might think to track the number of short-period gamma-ray bursts seen and to see whether their rates have changed over the Universe’s history. Unfortunately, there are fewer than 2000 gamma-ray bursts of all types ever detected, only about a quarter of them have redshift/distance measurements associated with them, and most of them are long or ultra-long gamma-ray bursts, not short-period gamma-ray bursts.

In other words, the data that we’ve collected, so far, shows no indication of an evolution in the neutron star-neutron star merger rate. However, it would be premature to rule this scenario out. Neutron stars, after all, arise as the corpses of massive stars that were born, burned through their fuel, and died in a cataclysmic supernova event. If two stars form close to each other with approximately the same mass, they’ll have approximately the same lifetime and can expect to undergo approximately the same fate. In other words, if you want to see neutron stars merge with other neutron stars, you should be looking at the environments where massive stars formed, lived, and died within the past few hundred million years.
But today, new stars are forming at a relative trickle compared to the deluge of star-formation earlier on in cosmic history. The star-formation rate today is only around 3% of what it was at the Universe’s peak: 10-to-11 billion years ago. It’s eminently plausible that, back when more stars were forming, more neutron stars in binary systems were forming and merging as well, and that the majority of our Universe’s, galaxy’s, and Solar System’s heaviest elements were formed very long ago, and it will take future observatories and more advanced technology to make that determination.
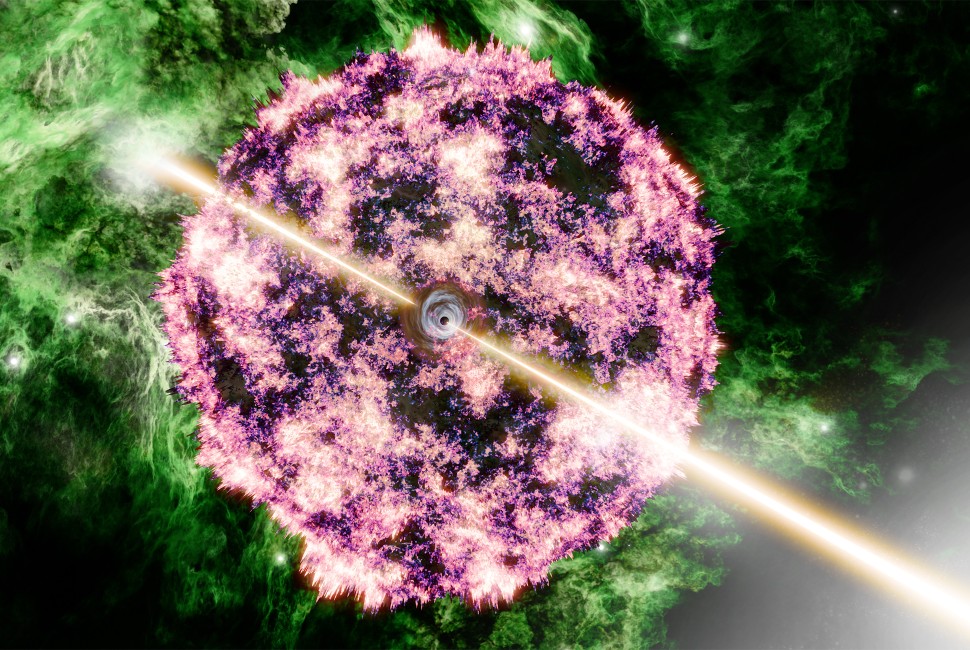
Scenario #2: the brightest, most energetic supernovae create large abundances of heavy elements.
Although it’s easy to group all types of heavy element production under the banner of “nuclear fusion,” the reality is a lot more complex. Nuclear fusion typically involves any light elements fusing together into heavier elements at great temperatures and densities through nuclear reactions. But these processes, typically occurring in the cores of stars, will only take you so far: up to elements such as iron, nickel, and cobalt, but no farther. Beyond that, it isn’t energetically favorable to fuse atomic nuclei together, so those reactions don’t occur spontaneously.
But you can continue to climb the periodic table by adding neutrons to your already-existing heavy elements, and core-collapse supernova events produce tremendous numbers of particles, and that possibly includes neutrons, in a very short period of time. As many astrophysicists have, you too might wonder whether the collapse of a sufficiently massive star could lead to the production of these heavy elements?
On October 9, 2022, astronomers detected the brightest gamma-ray burst of all-time, known simply as the B.O.A.T., and thought this would be a great testing ground, once the initial glow of the burst itself faded away, to see whether heavy elements were produced in the aftermath of this event or not. If so, it could potentially change how we perceived the suspected origin story of these heavy elements.
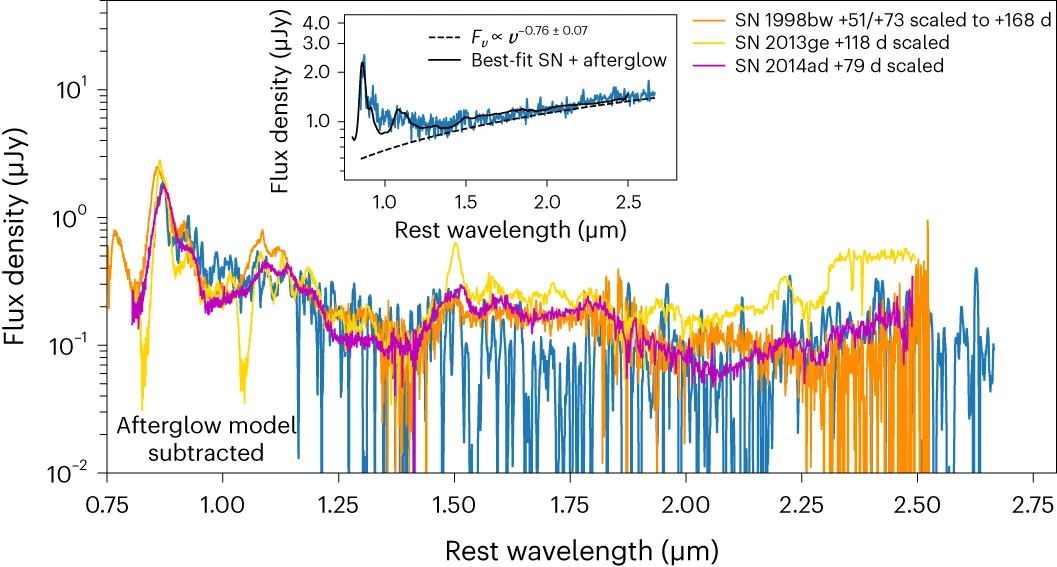
Using JWST data, a collaboration of some two dozen astronomers performed a spectroscopic analysis of the supernova remnant responsible for the brightest gamma-ray burst of all-time, and determined that the answer, quite definitively, is no. This gamma-ray burst, the brightest one ever observed, appears to have originated from a run-of-the-mill, mundane supernova after all. Whatever produced the gamma-ray burst part of this cataclysm — likely a combination of fast rotation, strong magnetic fields, and a tight collimation of emitted jets of radiation — doesn’t appear to have produced any notable quantities of heavy elements.
Instead, these follow-up observations suggested that normal supernovae:
- don’t produce large numbers of neutrons in rapid succession,
- don’t create enormous or even substantial quantities of very heavy elements,
- and don’t provide a key source of what astronomers call r-process material,
suggesting that supernovae that produce bright gamma-ray jets are not the culprit for creating the heavy elements in the Universe. It was a clever and reasonable idea to pursue, but now that the key evidence has come in, the verdict is that they do not. As study coauthor Ashley Villar put it,
“[S]ome had hypothesized that a luminous gamma-ray burst like the B.O.A.T. could make a lot of heavy elements like gold and platinum. If they were correct, the B.O.A.T. should have been a goldmine. It is really striking that we didn’t see any evidence for these heavy elements.”
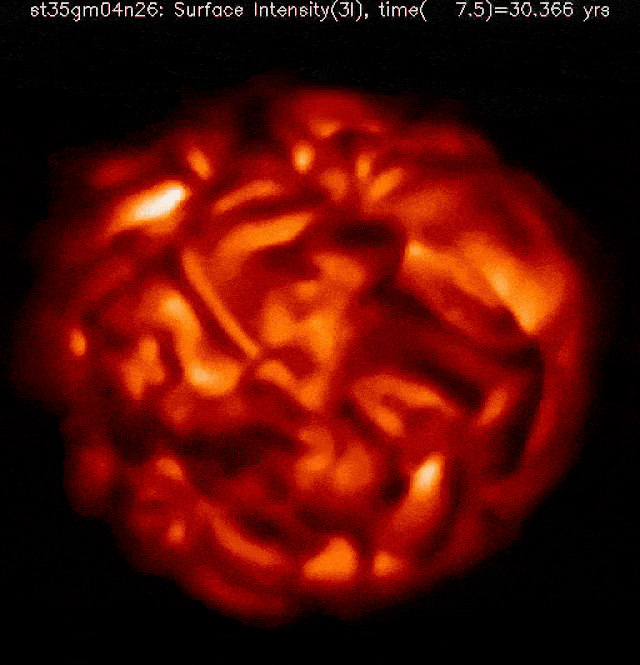
Scenario #3: normal, evolved stars create these heavy elements by slowly adding neutrons to pre-existing abundant elements.
In the cores of massive, evolved stars, however, fusion reactions occur that do frequently produce neutrons: over periods of many thousands of years, however, rather than all at once in a rapid burst. These neutrons, emitted primarily during carbon fusion and neon fusion, can be absorbed by any atomic nucleus — light ones, intermediate ones, or even heavy ones — and, if your new atomic nucleus is now too rich in neutrons, one of those neutrons will (beta) decay into a proton, bumping your atom up to a higher element number on the periodic table. In contrast to the rapid r-process, this slow neutron capture process is known as the s-process.
This process is well-understood and does occur in stars, and is responsible for a substantial fraction of the elements found to naturally occur: including tin, barium, some of the lanthanides, tungsten, mercury, and even lead. However, some elements are off-limits. If the new isotope would be more stable by “spitting out” a helium nucleus (an alpha decay process), it will do so spontaneously, preventing the formation of certain elements. Also, no elements heavier than bismuth can be created using this process, as when bismuth-209 captures a neutron, it first (beta) decays into polonium and then (alpha) decays back into lead, preventing the formation of the heaviest elements such as radon, radium, thorium, uranium and plutonium. This scenario creates some of the heavy elements, but it cannot account for them all.

When we put this all together, it means that we still have a deficiency in our understanding of how the heaviest elements in the Universe — from distant galaxies to our own planet — came to be. Evolved stars can only make a small fraction of them; supernovae don’t appear to make any in substantial quantities; and kilonova events, which are triggered by the merger of two neutron stars, aren’t frequent enough to explain the elements we observe. There must be something else at play beyond what is known today. While the “default” scenario might be to claim that the kilonova rate must have been much greater in the past than it is today, the most responsible stance to take, scientifically, is to state that we don’t know and must collect the critical data if we hope to find out.
Perhaps the most successful strategy would be to support the construction of a next-generation ground-based gravitational wave detector: one whose laser arms were approximately ten times longer than LIGO’s currently-running configuration with 4 kilometer arms. This could vastly increase the range at which neutron star-neutron star mergers could be observed: from hundreds of millions of light-years to billions of light-years, dramatically increasing our search volume as well as our capability of looking back in time to when the Universe’s star-formation rate was much greater. At present, however, we are forced to admit that our best pre-existing explanations for the origin of heavy elements, including gold, simply don’t add up. To find the best scientific answer, more work is needed.